Stars, Life and Society: Evolution in the Making
Almanac: Evolution: A Big History Perspective
My objective is to give a personal perspective on the history of the Universe, from the Big Bang to the origin of life on Earth, and life's evolution towards the enormous diversity that we witness today. My perspective is based in part on writing the college-level text The Universe and Life published in 1987, which – according to the founders of Big History – influenced the creation of this multi-disciplinary field. I begin by describing the circumstances that motivated me in the mid-1970s to start writing The Universe and Life. I then continue by giving a brief, updated summary of the text's content about physical and biological evolution; ask ‘Where Do We Go from Here?’; and discuss the philosophic and pedagogic challenges that I confronted. These are issues that characterize Big History as well. I conclude by commenting on some of Big History's unique challenges due to the field's broad, multi-disciplinary nature and suggest that we consider these as an opportunity to jointly move the field forward.
Introduction
Last September I received the preprint of an article ‘Changing the Future with the Past: Global Enlightenment through Big History’ by Barry Rodrigue and Daniel Stasko, both at the University of Southern Maine (Rodrigue and Stasko 2011). In the accompanying e-mail, Barry referred to me as ‘one of the legendary founders of Big History’ (pers. com., e-mail 5 Sept. Rodrigue to Kutter, 2010). I was rather surprised, to put it mildly.
I am an astrophysicist, but spent the latter part of my active career in administrative positions at the National Science Foundation, at NASA Headquarters, and in industry. Thus, I must admit that when I received Barry's e-mail I had not even heard of ‘Big History’! As I read on, I learned that the interdisciplinary text The Universe and Life: Origins and Evolution (Kutter 1987), which I wrote in the 1970s and 1980s, was the cause of his attribution.[1]
Barry and I have been friends since the early 1970s, when he was a student and I was a faculty member at The Evergreen State College, a small liberal arts college in Olympia, Washington (USA). Evergreen distinguishes itself by giving its students an interdisciplinary education via broadly designed programs taught by teams of faculty with diverse backgrounds. In addition, from its beginning in 1971, Evergreen lowered the traditional barriers between students and faculty by encouraging first-name interactions between them. It was in this informal but educationally rigorous setting that I got the idea to write The Universe and Life.
Barry serves as International Coordinator of the International Big History Association and invited me to write an article for a special edition of the almanac, Evolution, which is devoted to Big History. I felt honored by the invitation and accepted.
Motivation to Writing The Universe and Life
As I said, I am an astrophysicist. From the late 1960s to around 1990, my research focused on investigating the structure and evolution of stars via computer simulations – in particular the hydrodynamic events that stars of solar-mass experience as they age, such as pulsations and mass loss, either slowly and gently as planetary nebulae or suddenly and violently as nova explosions (selected references: Kutter and Sparks 1972, 1974; Sparks and Kutter 1987; Starrfield, Truran, Sparks, and Kutter 1972).
Upon arriving at The Evergreen State College in 1972, I team-taught with colleagues whose backgrounds were in chemistry, biology, geology, anthropology, the social sciences and the humanities. Once again, I became a student and began concerning myself seriously with fields beyond my own, learning from my colleagues and through seminar discussions with my students. This was the most fascinating, broadening and, yes, challenging education that a curious young PhD could hope for!
I began searching for the threads that tie diverse disciplines together. I asked myself: ‘What are the similarities and differences in the evolution of stars, galaxies and the Universe, on the one hand, and the evolution that we witness in the Solar System, in the geology of our planet, in the life that flourishes on it and, in particular, in our hominid lineage, on the other?’ I also asked: ‘How are these different evolutionary pathways related?’
These questions prompted me to research the relevant literature, develop written notes, and request teaching assignments on the subjects of physical and biological evolution, and the origin of life. Before long, I became totally consumed by this ‘Big History’ and began writing The Universe and Life.
I learned rather quickly that when we venture beyond our disciplines, we step into a minefield of potential misunderstandings, omissions and blatant errors. I had the good fortune that several of my Evergreen colleagues, in particular Burton Guttman and David Milne (both biologists), as well as Elizabeth M. Kutter (biophysicist, geneticist and founder of Evergreen's Laboratory of Bacteriophage Research), were willing to help me again and again when I had difficulties with concepts in their fields. They pointed me in the right direction when I went astray, and read and critiqued large parts of the manuscript. My wife, Sheryl Kutter (anthropologist), put up with my single-minded focus and was always ready for discussions, in addition to proof-reading the entire manuscript. I also got support from the College deans by giving me relevant teaching assignments and, towards the end of the project, a one-year leave of absence. Additionally, the publishers Jones and Bartlett solicited reviews of each of the chapters from recognized authorities in the respective disciplines.
Despite all of this support, Lynn Margulis, a biologist and professor at Boston University, wrote in the text's foreword: ‘I cannot help but notice that Kutter as a biologist still has some learning to do. I am sure that any anthropologist, zoologist, geophysicist, organic chemist, etc. will see small errors and differences in interpretation’. She then continued: ‘My response to this inevitability is that all of you specialists, students and teachers using this tome celebrate the magnitude of Kutter's effort by bringing to his attention your criticisms’.
Summary of the Content of The Universe and Life
The Universe and Life is divided into two parts: Physical Evolution and Biological Evolution.[2]
Part I. Physical Evolution
Long before the formation of the Solar System, a series of cosmic events took place that set the stage for the eventual origin of life on Earth and its evolution toward the enormous diversity we witness today. These events began roughly 13.7 billion[3] years ago when time and space, energy and matter (dark and baryonic[4]), as well as the laws of physics ‘emerged’ during one gigantic explosion, the Big Bang. Chaos reigned everywhere. Energy was largely in the form of high-energy electromagnetic radiation; and, after a brief period of primordial nucleosynthesis, baryonic matter consisted almost entirely of gaseous, ionized hydrogen and helium plus electrons. The heavier elements had not yet appeared. The radiation and matter were nearly homogeneously distributed throughout the Universe, except for very weak fluctuations in frequency distribution and density, respectively, which both had inherited from the Beginning – the so-called Planck Era. Furthermore, the radiation and baryonic matter were strongly coupled because the latter was ionized (radiation interacts with electric charges). The temperature was extreme.
The Universe was expanding furiously, due to the enormous amount of energy released during the Big Bang. Gradually, the expansion lowered the temperature; and by the time the Universe was roughly 400,000 years old, it had dropped to below 3,000 K. Now hydrogen and helium were able to capture electrons and keep them bound. Baryonic matter became neutral; and the initially strong coupling between it and radiation ceased. Radiation and matter decoupled. Each was now able to do its own thing.
Not much has happened to radiation since decoupling, except that its wavelengths got stretched by roughly a factor of 1,000 due to cosmic expansion. Today we observe it as the Cosmic Microwave Background (CMB) radiation. The CMB radiation is the same from every direction, except that it still possesses the weak fluctuations in frequency distribution.
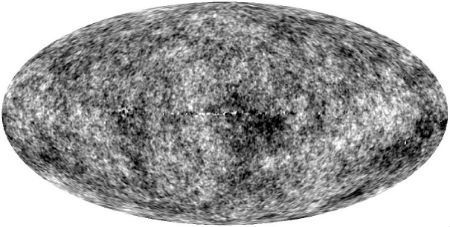
Fig. 1. All-sky map of the Cosmic Microwave Background radiation, the ‘oldest light’ in the Universe, dating to when the Universe was roughly 400,000 years old, and characterized today by a black body temperature of ~2.7 K. The radiation is isotropically distributed across the sky, except for weak fluctuations in temperature, amounting to about 1 part in 105. (Source: NASA / WMAP Science Team.)
Matter, too, followed its own evolution; but it has been far more interesting than that of radiation. Regions that had inherited above-average dark-matter densities possessed above-average gravity. They succeeded in locally slowing cosmic expansion and then began contracting via self-gravitation. Regions that had inherited lower-than-average densities had weaker gravity and, therefore, lost matter to higher-density regions. Baryonic matter followed these developments. In the course of some hundreds of millions of years, baryonic matter coalesced into the first generations of stars and proto-galaxies, followed by the formation of galaxies, galaxy clusters, and galaxy superclusters. Today, the galaxy superclusters and clusters form enormous walls, filaments, and bridges that crisscross the Universe, with huge voids between them. Some of these structures are many hundreds of millions of light-years in length (Bromm, Yoshida, Hernquist, and McKee 2009; Springel et al. 2005).
In the cores of the newly formed stars, nuclear reactions fused hydrogen and helium into heavier elements, such as carbon, nitrogen, oxygen, silicon, etc. As the most massive stars reached the ends of their lives, they exploded as supernovae and ejected newly synthesized elements into interstellar and intergalactic space. There, the elements intermingled with primordial hydrogen and helium. From this enriched mix new generations of stars were born, some of which also exploded and further contributed to the gradual buildup of heavy elements in the Universe.
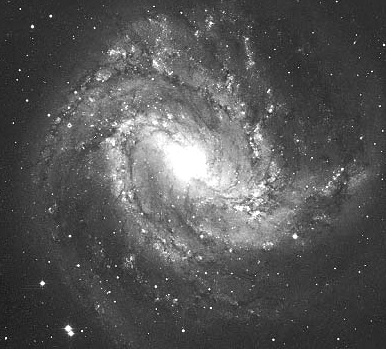
Fig. 2. The Southern Pinwheel galaxy, Messier 83, at a distance of ~15,000 light-years. If we could view our galaxy, the Milky Way, from afar, it probably would resemble M 83, with a similar, bar-like central bulge and graceful spiral arms; plus star-forming regions, bright nebulae lit up by recently formed stars, and dark, light-absorbing dust lanes. (Source: S. Lee, C. Tinney, D. Malin, and the Australian Astronomical Observatory.)
Among the billions of galaxies populating the Universe, one was the Milky Way. In an interstellar cloud, located in one of its spiral arms and enriched with heavy elements, the Sun and its planets formed approximately 4.5 billion years ago.[5] The third planet from the Sun was Earth. During its formation, Earth lost most of its hydrogen, helium, and other volatiles. What remained was a gravitationally differentiated globe consisting of an iron-nickel core surrounded by a rocky, silicate-rich mantle and, initially, a magma ‘ocean’. As the Earth cooled, this ocean hardened into a solid crust, probably within the first 100 to 200 million years of its existence (Hawkesworth and Kemp 2006).
During the first billion years, Earth and the other terrestrial planets were repeatedly bombarded by bodies, large and small (some were 1000 km and larger in size), called planetesimals. Their equivalents today are asteroids and comets. In fact, the Moon is thought to have been formed when a planetesimal roughly the size of today's Mars impacted on the nearly formed Earth, some 30 to 50 million years after the origin of the Solar System. Some of the material blasted into space by the giant impact went into orbit around the Earth and accreted to form the Moon. Impact scars from the later phases if this interplanetary bombardment, the so-called period of late heavy bombardment, between roughly 4.1 and 3.8 billion years ago, are still in evidence today on the surfaces of such geologically inert bodies as Moon and Mercury[6] (Kleine et al. 2005).
The cometary planetesimals came from the outer regions of the Sun's planetary system, probably perturbed in their orbital paths by Jupiter and the other giant planets. These cometary bodies consisted largely of water ice and frozen volatiles rich in biogenic elements, such as carbon, nitrogen, oxygen, phosphorus, sulfur, and other elements, as well as simple organic molecules, necessary for the eventual origin and evolution of life. These freshly delivered materials covered the Earth with oceans and an atmosphere.[7] From the Sun, Earth received a steady supply of radiation, which provided warmth and light.
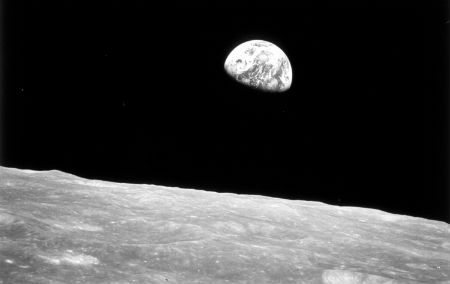
Fig. 3. Earth as seen from NASA’s Apollo 8 Command Module, orbiting the Moon 110 km above its impact-scarred surface (December 1968)
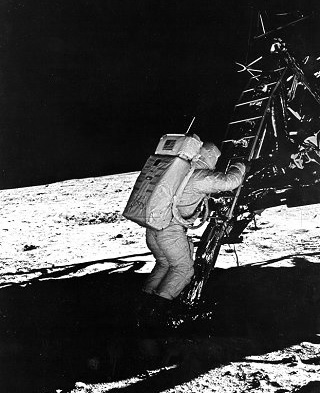
Fig. 4. The first landing by humans on a planetary body beyond Earth (Apollo 11, July 20, 1969), attesting to our spe-cies’ indomitable spirit of exploration and adventure
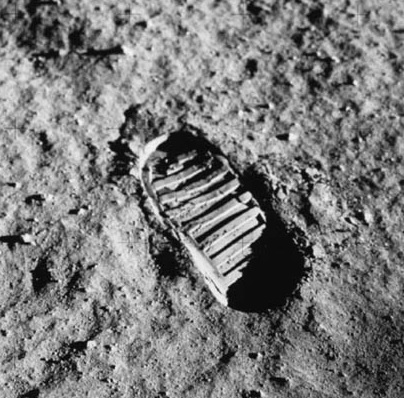
Fig. 5. Human footprint in Lunar soil (Source: Figs 3, 4, 5 NASA/ Apollo Program)
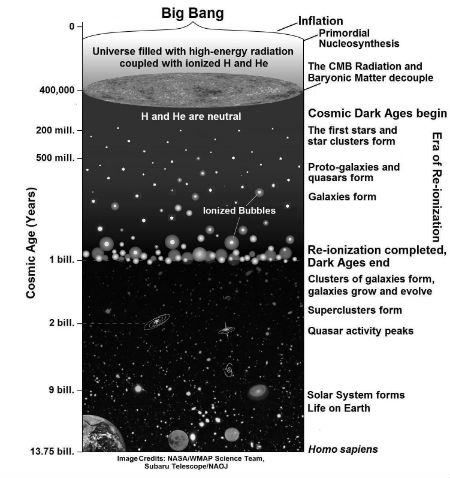
Fig. 6. Major events in the physical evolution of the Universe – from the Big Bang to today (Source: National Astronomical Observatory of Japan, Subaru Telescope, Press Release, 24 Feb. 2009.)
Part II. Biological Evolution on Earth
As the young Earth acquired, via cometary bombardment, an ocean consisting of water, along with biogenic elements, we may assume that locally conditions arose that were well suited for the occurrence of a multitude of different kinds of chemical reactions. Many of these reactions were probably driven by such energy sources as heat from lava eruptions, lightning, shocks from impact events, and solar UV radiation, and catalyzed by the physical environment (e.g., mineral surfaces). The result was the creation of a great variety of simple organic molecules. Over time, the molecules became larger and more complex. Amino acids, nucleic acids, sugars, fatty acids, and other small organic molecules, and polymers composed of them accumulated in the ocean, became locally concentrated, and created what is often referred to as the ‘prebiotic soup’.[8]
The interactions between the molecules increased in diversity and eventually some sort of order emerged. This order must have included the first primitive means of both storing information and carrying out catalysis, functions required for biological reproduction that today are performed by deoxyribonucleic acids (DNA) and proteins, respectively. It has been suggested that early on this dual function was performed by a polymer that is related to but predates DNA, namely ribonucleic acid (RNA) or a precursor of RNA. However, to date attempts to experimentally create such a polymer under prebiotic-soup-conditions (i.e., abiotically) and thus to confirm the so-called ‘RNA world hypothesis’ have not been successful (Powner, Gerland, and Sutherland 2009). In any case, according to our current understanding, within possibly a few 100 million years of the formation of Earth, the initially relatively simple chemical and physical interactions led to the origin of self-maintaining chemical systems – the first protocells on Earth.
Of course, there may have co-existed numerous different ‘species’ of protocells, competing with each other, probably inhabiting a range of different environments, and co-evolving. Furthermore, ‘species’ of protocells may periodically have become extinct when large planetesimals impacted on Earth, particularly during the period of late heavy bombardment; and new ‘species’ arose subsequently. But based on the biochemical and structural similarities of modern cells, it is believed that all of today's life evolved from a single protocell lineage – the last universal common ancestor or LUCA. The other protocell lineages died out (Forterre and Gribaldo 2007).
The LUCA cells lived in the juvenile ocean and resembled today's bacteria, though they probably were considerably less complex. They were capable of neither photosynthesis nor respiration, but obtained their nourishment by the anaerobic process of fermentation of organic molecules synthesized in the soup. Furthermore, they did not reproduce sexually, by the mating of males and females, but by direct asexual cellular division. However, natural selection – the maintenance of genetic diversity and the preferential survival of those individuals best adapted to the prevailing conditions in the environment – exerted itself right from the beginning. Over time, the LUCA cells became more complex; metabolic pathways became more efficient and versatile (e.g., photosynthesis developed); and by roughly 2 billion years ago, the three domains of modern cells had evolved: the bacteria and archaea, which are prokaryotes (cells without nuclei), and the eukarotes. Subsequently, the eukaryotes evolved into protists, fungi, plants, and animals, including humans.[9], [10]
The human phylogenetic lineage goes back about 5 to 7 million years in Africa to the last common ancestor of today's humans and chimpanzees. That ancestor was an arboreal animal who lived in the tropical forest. For roughly one million years after this speciation, our early ancestors retained anatomical features that allowed them to live as both climbers in the arboreal environment and upright bipeds on the ground. Gradually, as the climate became drier and more seasonal, our ancestors began moving from the receding forest out into the grasslands of the surrounding savannah; and their dual adaptation shifted more and more toward habitual bipedalism. It was an adaptation that allowed them to walk over great distances and to quickly escape predators. An example of this adaptation is found in fossil records of the australopithecines (meaning ‘southern apes’), who appeared around 4.2 million years ago and survived for close to three million years.
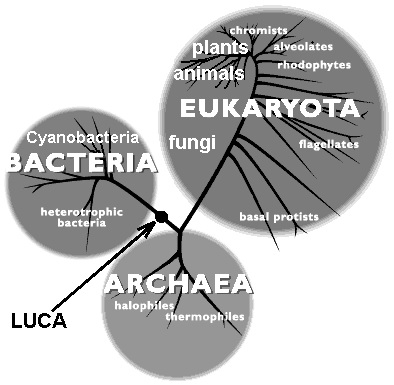
Fig. 7. The phylogenetic tree (simplified) showing the three domains of terrestrial life: Bacteria, Archaea, and Eukaryota. The organisms of the three domains are thought to have evolved from a single ‘last universal common ancestor’ or ‘LUCA’ (Source: Carl Woese, University of Illinois.)
Roughly 2.4 million years ago the genus Homo evolved from the australopithecines; and by 250,000 to 200,000 years ago H. sapiens (meaning ‘wise’ man) or modern man appeared, still in Africa, who possessed a large brain, language, sophisticated stone tool technologies, and fire, and, we may assume, was capable of abstract thought and developed social structures and culture. Earlier members of the genus Homo, such as H. habilis, H. erectus, and several other Homo species possessed some of these characteristics, too, but not to the same degree.[11] By about 100,000 to 50,000 years ago – the timing is currently a matter of considerable debate among physical anthropologists – tribes of H. sapiens migrated out of Africa and began spreading across the globe.
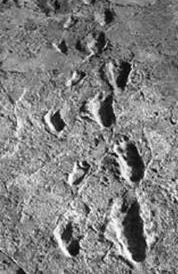

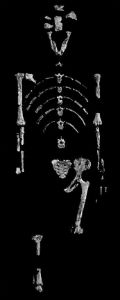
Fig. 10. Fossil hominid, Lucy
Like the Laetoli people, ‘Lucy’ was a member of Australopithecus afarensis and lived roughly 3.2 million years ago in today’s Hadar region of Ethiopia.
The fossil skeleton was found in 1974 by the international Afar Research Expedition, whose members named her after the then popular Beatles song ‘Lucy in the Sky with Diamonds’.
The skeleton, which is nearly 40 % complete, indi-cates that Lucy was an adult female about 25 years old who, like her contemporaries, was rather small – a little more than one meter in height, with a weight of about 30 kg. The ratio of the length of her arms to that of her legs is less than that of modern chimpanzees, but greater than that of mo-dern humans, suggesting that even though Lucy was a habitual biped, she still retained some arboreal adaptations. (Source: Cast from the Museum national d'histoire naturelle, Paris.)
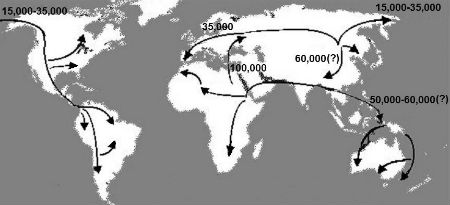
Fig. 11. Major human migration paths out of Africa and across the globe, with estimates of their timing (years ago) [12] (Source: Cavalli-Sforza, Menozzi, and Piazza 1994.)
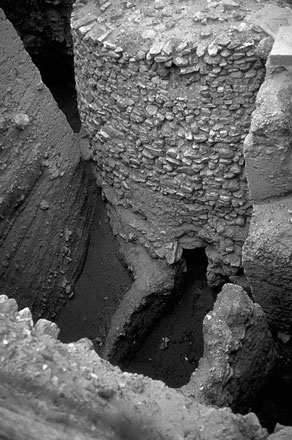
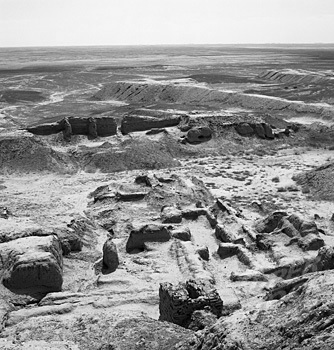
Early human populations were hunters and gatherers. They lived off the land, eating the fruits, grains, and vegetables that were in season and following herds of wild animals in their yearly migrations. But by about 10,000 years ago, as the last ice age was coming to a close and the climate grew milder, some groups of H. sapiens were beginning to cultivate plants and domesticate animals; and they began building villages and (later) towns. They were gaining independence from the vagaries of nomadic life. The earliest known signs of human settlements and an agricultural way of life are found in the Fertile Crescent of the Middle East, which arches from the Jordan Valley and Syria through eastern Turkey to Iraq and Iran. Here we find stone ruins of houses, walls, and watch towers nearly 10,000 years in age. Examples are Jericho and (later) Uruk. Jericho, on the west side of the Jordan River valley, had a more than 5000-year-old history when biblical writers made reference to it, and at one time is thought to have had a population 2000 to 3000. Uruk (Erech in the Bible) was located on the banks of the Euphrates River in today’s Iraq and was one of the principal cities of ancient Sumer. It was enclosed by brick walls about ten kilometers in circumference, built by the mythical king Gilgamesh. At the height of its development by around 2900 BC, the city is estimated to have had a population of some 50,000.[13] (Source: Figs 12, 13, Casa Editrice Scode, S.p.A., Italy.)
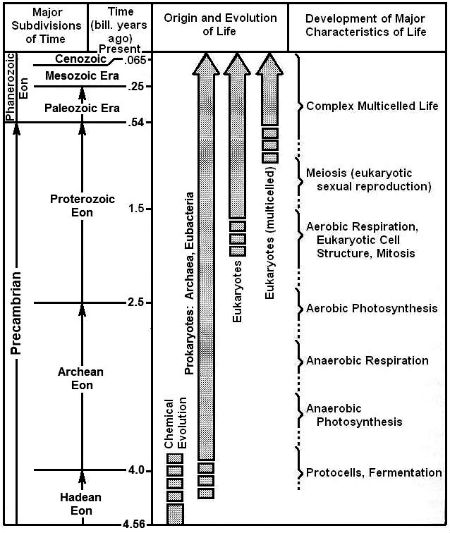
Fig. 14. Major events in biological evolution on Earth (Source: Kutter 1987, updated.)
Where Do We Go from Here?
The images below illustrate the huge divide that exists today between the subsistence living of people in the underdeveloped world, on the one hand, and the materialistic life styles, wanton pollution of the environment, and excessive military spending in at least parts of the industrialized world, on the other. Unless we, the people of the Earth, manage to narrow this divide; take the wide-spread degradation of the environment, including the human contribution to global warming, seriously and begin counteracting it; preserve the planet's biodiversity; recognize that the world is finite, which places limits on population, economic growth, and availability of natural resources; reduce military spending and weapons arsenals; and learn to live in harmony with our environment and with each other; humankind will surely be marching toward an abyss.
In my view, to avoid a catastrophe, we must set aside our prejudices; make use of our scientific and technological knowhow; and, as I wrote in The Universe and Life, ‘we must learn to combine the two seemingly contradictory attitudes – modern man's reliance on science and technology and the nomad's maxim of adaptation to the environment’ (O'Neill, Dietz, and Jones 2010; Victor 2010; Kutter 1987: 546).
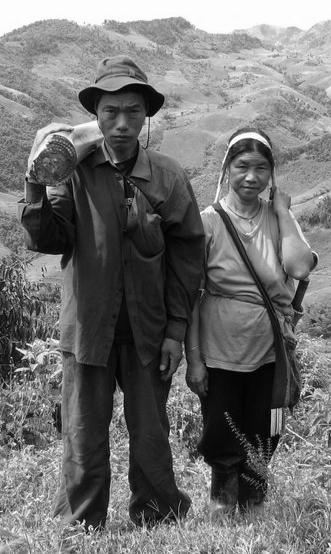
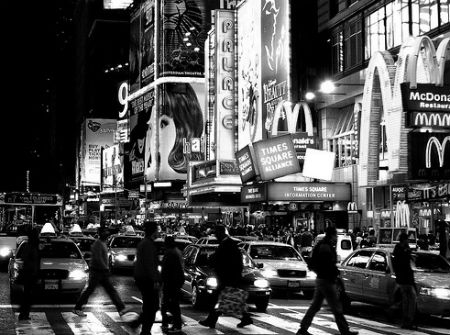
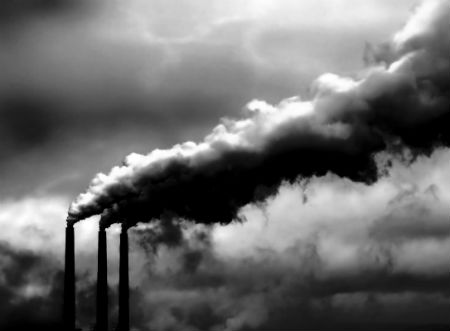
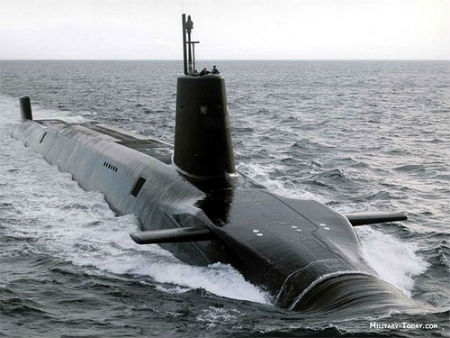
Physical vs. Biological Evolution
According to our current scientific understanding, the evolution of the inanimate Universe is governed by the laws of physics that humans have deduced, over the course of centuries, via experiments in terrestrial laboratories and by observing events in the Solar System (e.g., orbital motions, star light making grazing passages of the Sun's limb) and the Universe beyond.
On macroscopic scales, the classical laws of physics from Newton's mechanics; Carnot's, Joule's, Clausius', and Lord Kelvin's thermodynamics; Faraday's and Maxwell's electricity and magnetism; etc. to Einstein's theories of special and general relativity are deterministic. This means that if the initial state of an isolated system is known, the laws allow a competent theoretician with sufficient computer power to predict all future states of the system.
On the microscopic scales of molecules, atoms and elementary particles, Heisenberg's, Born's and Schrödinger's quantum mechanics rule. Quantum mechanics is a probabilistic theory because there are limits to how well the state of a microscopic system can be known, today or in the future. The probabilistic nature of quantum mechanics is embodied in Heisenberg's uncertainty principle and confirmed by measurements, according to which it is impossible to determine simultaneously and with arbitrary precision both the position and momentum of electrons, protons and other particles.
These laws – both classical and quantum mechanical – are consistent with nature's conservation laws: the conservation of mass-energy, linear momentum, angular momentum, electric charge, and several other physical characteristics that evidence themselves on the sub-atomic level.
Moving on now to biology, we believe that all of its processes – from the biochemical and physical processes of individual organisms to biological evolution – are also consistent with the laws of physics, because organisms are made of the same kind of baryonic matter that we find in the inanimate Universe. But even though organisms and their internal processes, as well as their interactions with their environments, are subject to the laws of physics, they do distinguish themselves from inanimate objects in a number of fundamental ways:
1) Organisms possess unique structural organizations – on both microscopic and macroscopic scales – that are enormously complex and give rise to a range of physical processes more or less optimized for the organisms' survival and reproduction. It is these organizations and processes that give them the quality we call living.
2) Organisms cannot survive on their own. They all are dependent on and part of ecosystems, typically consisting of a great many components, both biotic and abiotic, as well as ranges in size, that dynamically interact with each other in a multitude of ways and via complex feedback loops. Nothing quite comparable is found in the inanimate world.
3) However, the most profound difference between organisms and inanimate objects is the fact that the former reproduce according to genetic information encoded in long molecular strands – deoxyribonucleic acids or DNA. Inanimate objects do not. Over time, the genetic information of members of a population changes in a two-step process of chance and selection:
In the first step, random mutations and their recombinations gradually alter the genetic information of a population from generation to generation, thereby contributing to and maintaining a large degree of variation in the genetic makeup among the population's individual members.
The second step results from the fact that some members of a population are, because of their particular genetic makeup, more competent – due to biochemical, anatomical, and/or behavioral traits – than others in finding food and shelter, defending against predators, resisting disease, and dealing with changes in climate and geophysical conditions. These more competent members are more likely than others to survive, reproduce and pass their genetic makeup on to the next generation.
This inequality among members of a population in the likelihood of passing on genetic information constitutes a selection process. It continually pushes the genetic makeup of a population in directions that better adapts them to changing biological and climatic/geophysical environments and increases the likelihood of survival of the population. The result is Evolution by Natural Selection, as first recognized independently in 1858 by two British naturalists, Charles Darwin and Alfred Wallace.
This two-step process of chance and selection is also the driver of speciation, the evolutionary process by which new biological species arise.
Philosophic and Pedagogic Challenges
In writing The Universe and Life, I used the chronologic approach, which presented two challenges that characterize Big History as well – one philosophic, the other pedagogic.
The philosophic challenge arose from the chronologic order of the presentation of the topics, which might mislead the reader into thinking that there is purpose to evolution, namely the making of humankind. This view is not warranted, at least as far as is known from science. The laws of nature came into being, along with time and space, energy and matter, during the Big Bang, and they have governed the evolution of the Universe and all it contains ever since. The appearance of life on Earth, including humankind, is a consequence of these laws, just as the formation of stars and their planetary systems, galaxies, and galaxy clusters is. Science does not accord any special status to life or to humankind. This conclusion had already been reached by 17th century philosopher Benedict de Spinoza, when he wrote in his influential metaphysical treatise Ethica: ‘Nature has no particular goal in view, and final causes are only human imaginings’.
The pedagogic challenge arose from the fact that the Universe and Life is written on the introductory level, yet, because of its chronologic approach, starts with complex and difficult topics. For example, Chapter One deals with the Big Bang, during which conditions were far from simple and as different from our experiences as can be imagined. Likewise, theories of the emergence of the earliest organisms on Earth and the eventual evolution of some of them toward complex multi-celled life based on the eukaryotic cell structure, aerobic respiration, and sexual reproduction are topics with which most students are probably not familiar, and that to a considerable degree are still controversial. Clearly, a chronologic approach did not allow me to start with the familiar and simple and then develop the subject matter in a systematic manner, as is common in traditional texts.
In order to minimize the difficulties that a chronological approach presents, I wrote introductions to Parts I and II that establish essential background information. Furthermore, each chapter contains numerous comments, short essays, and tables that are boxed to set them off from the main text. They offer additional information that, if included in the text, would interrupt the flow of the presentation.
Looking back today, from the perspective of more than two decades since the publication of The Universe and Life, I recognize yet another challenge, or put more positively, an opportunity, which at the time I did not appreciate fully. This opportunity reveals beauty, as well as new insights to the subject of Big History. It was articulated by the English sculptor Henry Moore, when he was asked by Nobel Prize winning Indian-American astrophysicist Subrahmanyan Chandrasekhar: ‘How should one view sculptures: from afar or from nearby?’ Henry Moore replied: ‘The greatest sculptures can be viewed – indeed should be viewed – from all distances, since new aspects of beauty will be revealed on every scale’ (Chandrasekhar 1979).
Likewise, I believe that the Universe and its contents should be viewed and studied on all scales accessible to our telescopes and, here in our Solar System, more directly through space missions; and so should terrestrial life, by direct observations and with the aid of microscopes, from the scale of ecosystems to that of individual organisms, cells, viruses, and their contents. At every scale we will find new insights, surprises, and beauty, and new interconnections and complexities.
Conclusion
I would like to conclude by asking you to re-read Lynn Margulis' words above, pointing out that, ‘I as a biologist still have some learning to do’, and then suggesting that the ‘specialists, students, and teachers using the text bring to my attention their criticisms’. In this spirit, I would like to propose that we, who are participants in the development and teaching of Big History, recognize that our intellectual endeavour is so immensely broad that no single individual or even members of a single academic institution can likely be experts in all that the field entails.
As we advance Big History, ‘errors and differences in interpretation’ are inevitable. But let us not be disheartened by this fact. Instead, let us use the challenges of Big History as our guiding principle to generously communicate with each other; collaborate and constructively critique each others' contributions, particularly at the interfaces of different disciplines; and patiently, but resolutely, move the field forward toward the common goal: ‘…to understand, in a unified and interdisciplinary way, the history of Cosmos, Earth, Life, and Humanity’ (see International Big History Association website at http://www.ibhanet.org/).
Aknowledgement
I would like to thank Dr. Mark E. Kowitt, Dr. Elizabeth M. Kutter, Dr. Peter D. Noerdlinger, and my wife, Sheryl, for critically reading the manuscript and making numerous valuable comments and corrections; and Dr. Barry Rodrigue for editorial improvements.
[1] Some of the citations for Dr. Kutter as a forerunner of Big History can be found in Christian 2004; Spier 2010.
[2] The following descriptions of physical and biological evolution are in part based on sections from the preface of The Universe and Life, but have been expanded and updated to reflect current understanding.
[3] In this paper I am using the US convention for billion: One billion = 1000 million = 109.
[4] Baryonic matter is the kind of matter we, the Earth, the Sun and the rest of the visible Universe are made of. In contrast, dark matter cannot be seen. It makes itself felt only via the gravitational effect it has on baryonic matter and electromagnetic radiation. To date dark matter has not been detected on scales of planets and stars, only on scales of galaxies and larger structures. Dark matter's mass exceeds that of baryonic matter by roughly a factor of five.
In addition to baryonic and dark matter, cosmologists hypothesize that the Universe is permeated by an energy they call dark energy. Dark energy differs from the energy associated with baryonic matter, such as kinetic energy, potential energy (e.g., gravitational), chemical energy, heat energy, etc. Dark energy is thought to be a property of space itself and postulated to be responsible for the observed current acceleration of cosmic expansion. Interpretations of the cosmic microwave background (see Fig. 1) suggest that today dark energy amounts to ~72.8 % of the total mass-energy of the Universe, while baryonic and dark matter amount to ~4.6 % and ~22.7 %, respectively (Jarosik 2011).
[5] The interstellar matter from which the Sun and its planets formed consisted approximately of 71 % hydrogen, 27 % helium, and 2 % heavier elements (by mass).
[6] The bombardment of Earth by interplanetary debris continues today, but at a much reduced rate. Most of today's bodies, arriving from interplanetary space, are extremely small compared to those early in our planet's history. The vast majority is roughly of the size of sand grains, though some have dimensions comparable to boulders. We call them meteors or shooting stars when they pass as fiery streaks across the night sky, or, if they are very bright, bolides.
[7] The early terrestrial ocean and atmosphere were anoxic. Molecular oxygen began accumulating on Earth only after the appearance of cyanobacteria, the earliest known aerobic (O2-releasing) photosynthesizers, around 2.8 billion years ago. The oxygen level in the atmosphere began increasing measurably about 2.3 billion years ago (Olson 2006).
[8] There currently exist two principal theories for the origin of life on Earth – the prebiotic soup theory (a cold, oceanic origin) and the autotrophic metabolic theory (a hot origin, mainly energized by geothermal sources). The former theory links early terrestrial biochemistry with the preceding cosmic chemistry; while the latter links it with geochemistry. In the above description of how life might have originated on Earth, I favour the prebiotic soup theory, though I also took some elements (e.g., catalysis on mineral surfaces) from the autotrophic theory (Bada et al. 2007).
[9] In addition to archaea, bacteria, and eukaryotes there exist viruses, which are microscopic parasites possessing genes, but by themselves cannot reproduce. Therefore, traditionally they have not been classified among living organisms. Viruses reproduce by infecting appropriate host cells and subverting their reproductive machinery to produce offspring viruses. Recent research has shown that viruses probably have played and are still playing major roles in the evolution of cellular organisms; and their place in the universal tree of life has become an active topic of discussion.
[10] It was the accumulation of molecular oxygen (O2) in the terrestrial ocean and atmosphere that made possible the evolution of eukaryotes, which are obligatory aerobes. Molecular oxygen offered the potential of developing metabolisms that combine oxygen and hydrogen (the latter typically obtained from organic materials, such as sugars and fats) to form water (H2O), a reaction that liberates far more energy than anaerobic reactions. However, O2's high reactivity makes this molecule potentially lethal to life. Two evolutionary ‘inventions’ were required to counteract this danger. First, ‘escort’ molecules had to evolve that bind with O2 and make it harmless while circulating within the cytoplasm of cells. Second, metabolic pathways needed to evolve that ensure that the reaction of O2 + 2 H2 Þ 2 H2O does not occur suddenly (as it does, for instance, in burning wood), but in a series of small steps, so that the reaction energy is released gradually and, hence, safely. The evolution of cyanobacteria, the first aerobic photosynthesizers on Earth, was accompanied by both inventions. All subsequent aerobic life adopted, with various adaptations and improvements, this two-step strategy of the early cyanobacteria, allowing evolution to take the great multitude of paths it has and creating the enormous biodiversity that characterizes our planet today.
[11] Our closest relatives were the now extinct members of H. neanderthalensis, with whom we share a last common ancestor that goes back about 500,000 years in Africa. In fact, some anthropologists think that the Neanderthals were not a separate species from us, but a subspecies – Homo sapiens neanderthalensis. Early on, the Neanderthals left Africa and settled in Asia and Europe, where they survived until about 50,000 and 30,000 years ago, respectively. Genetic evidence indicates that humans and Neanderthals interbred about 60,000 years ago in the Eastern Mediterranean and, more recently, about 45,000 years ago, in eastern Asia. Of course, there probably were other interbreeding events that we may never know about. As a result, approximately 1 to 4 % of the genome of Eurasian people derives from H. neanderthalensis; but to date, no evidence exists of interbreeding in the genomes of African people (Dalton 2010).
[12] This map gives a good overview of human migration paths, but is slightly dated. For a more up-to-date and detailed map see: National Geographic Maps, Atlas of the Human Journey, URL: http://www.utexas.edu/features/2007/ancestry/graphics/
[13] It is worth noting that protective city walls and towers have a long history, signifying that conflicts, wars and conquests go back a long way and attesting to the aggressive nature of our species.
Bada J. L., Fegley B. Jr., Miller S. L., Lazcano A., Cleaves H. J., Hazen R. M., and Chalmers J. 2007. Debating Evidence for the Origin of Life on Earth. Science 315: 937–939.
Bromm V., Yoshida N., Hernquist L., and McKee C. F. 2009. The Formation of the First Stars and Galaxies. Nature 459: 49–54.
Cavalli-Sforza L. L., Menozzi P., and Piazza A. 1994. The History and Geography of Human Genes. Princeton: Princeton University Press.
Chandrasekhar S. 1979. Beauty and the Quest for Beauty in Science. Physics Today 63(12): 57–62.
Christian D. 2004. Maps of Time. Berkeley, MA: University of California Press.
Dalton R. 2010. Neanderthals May have Interbred with Humans. Nature. DOI: 10.1038/news.2010.194
Forterre P., and Gribaldo S. 2007. The Origin of Modern Life. HFSP Journal 1(3): 156–168.
Hawkesworth C. J., and Kemp A. I. S. 2006. Evolution of the Continental Crust. Nature 443: 811–817.
Jarosik N., Bennett C. L., Dunkley J., Gold B. et al. Seven-Year Wilkinson Microwave Anisotropy Probe (WMAP) Observations: Sky Maps, Systematic Errors, and Basic Results. The Astrophysical Journal Supplement Series 192: 2–14.
Kleine T., Palme H., Mezger K., and Halliday A. N. 2005. Hf-W Chronometry of Lunar Metals and the Age and Early Differentiation of the Moon. Science 310: 1671–1674.
Kutter G. S. 1987. The Universe and Life: Origins and Evolution. Cambridge: Jones and Bartlett Publishers, Inc.
Kutter G. S., and Sparks W. M. 1972. Studies of Hydrodynamic Events in Stellar Evolution. I. Method of Computation. The Astrophysical Journal 175: 407–415.
Kutter G. S., and Sparks W. M. 1974. Studies of Hydrodynamic Events in Stellar Evolution. III. Ejection of Planetary Nebulae. The Astrophysical Journal 192: 447–455.
Olson J. M. 2006. Photosynthesis in the Archean Era. Photosynthesis Research 88: 109–117.
O'Neill D. W., Dietz R., and Jones N. 2010. (Eds.) Enough is Enough: Ideas for a Sustainable Economy in a World of Finite Resources. The report of the Steady State Economy Conference. Leeds: Center for the Advancement of the Steady State Economy and Economic Justice for All. URL: http:\\www.steadystate.org/enough-is-enough/. Date accessed: 12.03.2011.
Powner M. W., Gerland B., and Sutherland J. D. 2009. Synthesis of Activated Pyrimidin Ribonucleotides in Prebiotically Plausible Conditions. Nature 459: 239–242.
Rodrigue B., and Stasko D. 2011. Changing the Future with the Past: Global Enlightenment through Big History. The Journal of Globalization Studies 1(2): 30–47.
Spier F. 2010. Big History and the Future of Humanity. Oxford: Wiley and Blackwell.
Sparks W. M., and Kutter G. S. 1987. Nuclear Runaways in a C/O White Dwarf Accreting H-Rich Material Possessing Angular Momentum. The Astrophysical Journal 321: 394–403.
Springel V., White S. D. M., Jenkins A., Frenk C. S. et al. 2005. Simulations of the Formation, Evolution and Clustering of Galaxies and Quasars. Nature 435: 629–636.
Starrfield S., Truran J. W., Sparks W. M., and Kutter G. S. 1972. CNO Abundances and Hydrodynamic Models of the Nova Outburst. Nature 176: 169–176.
Victor P. 2010. Questioning Economic Growth. Nature 468: 370–371.